Logic level MOSFETs have typical values of about 2V to 3V, whereas other devices can have higher values. 6-4 the threshold voltage is plotted against junction temperature. The datasheet specifies the typical threshold as 1.8 V for a drain current of 250 µA, which puts the MOSFET in.
- A resistive load, R2 (2 Ω), is used to test the circuit. R1 is adjusted to set the voltage close to the threshold voltage of Q1, which is measured as approximately 1.9 V. With the TPL191B, the.
- I am using several MOSFETs (STP16NF06 datasheet here) controlled by a shift register (74HC595 datasheet here) which is controlled by a microcontoller. The spec sheet says the gate threshold voltage is between 2V and 4V. When controlling directly via the MCU, the voltage on the pin is 3.3V. However, I have switched to a shift register (to save pins) which is connected to the same 5V source that the.
A correctly calculated MOSFET turn-ON process ensures that the device is switched ON with optimal efficiency.
While designing MOSFET based circuits you might have wondered what is the correct way of turning ON a MOSFET? Or simply what is the minimum voltage that should be applied across gate/source of the device to switch it ON perfectly?
Although for many digital systems this might not be an issue, 5V systems such as DSPs, FPGAs, and Arduinos require boosting of their outputs for optimal switching condition for the connected MOSFET.
And in these situations the designer begins looking at the specifications of the MOSFET to get the threshold voltage data. The designer assumes that the MOSFET would turn ON and change state when this threshold level is crossed.
However this may be not as simple as it may appear to be.
What is Threshold Voltage VGS(th)
First of all we must realize that the threshold voltage, denoted as VGS(th) is not for circuit designers to worry about.
To be precise, it is the gate voltage which causes the drain current of the MOSFET to cross a threshold level of 250 μA, and this is tested under conditions that might never normally transpire in practical applications.
During certain analysis, a constant 5V is used for the above mentioned testing of the device. But this test is normally implemented with the gate and the drain of the device connected or shorted with each other. You can easily get this information in the datasheet itself, so there's nothing mysterious about this test.
The table above indicates the threshold levels and the relevant test conditions for an example MOSFET.
For a desired application the designer might be worried about a dreaded situation known as 'induced' gate voltage, which may be a serious issue for example in a low side MOSFET of synchronous buck converter.
As discussed earlier, here too we must understand that crossing the threshold VGS(th) level may not force the device to run into a shoot-through breakdown condition. This level actually tells the designer regarding the threshold at which the MOSFET just begins turning ON and is not a situation where things just end altogether.
It may be advisable that while the MOSFET is in the switched OFF condition the gate voltage is maintained below the VGS(th) level , to prevent current leakage. But while turning it ON this parameter may be simply ignored.
Transfer Characteristic Curve
You will find another curve diagram named transfer characteristics in MOSFET datasheets explaining its turn ON behavior in response to increasing gate voltage.
To be precise this may be more related to current variation analysis with respect to gate voltage and device case temperature. In this analysis the VDS is held at a fixed level but high level, around 15V, which may not be revealed in the datasheet specs.
If we refer to the curve as shown above we realize that for 20 Amp drain current, 3.2 V gate-to-source voltage may not be adequate.
The combination would result in a VDS of 10 V typically with a dissipation of 200 watts.
Transfer curve data can be useful for MOSFETs operated in the linear range, however the curve data may have less significance for MOSFETs in switching applications.
Output Characteristics
The curve which reveals the actual data regarding the fully ON condition of a MOSFET is known as the output curve as shown below:
Here, for the various levels of VGS the forward drop of the MOSFET is measured as a function of current. Device engineers use this curve data to confirm the optimal level of gate voltage.
For each level of gate voltage that ensures a full switch ON of the MOSFET [RDS(on)], we get a range of voltage drops (VGS) across drain-to-source having strictly linear response with drain current. The range begins from zero and upwards.
For lower gate voltages (VGS), when the drain current is increased, we find the curve losing the linear response, moving through the 'knee' and then going flat.
The above curve details provide us the complete output characteristics for a range of gate voltages from 2.5 V to 3.6 V.
MOSFET users may normally contemplate this as the linear function. However, in contrast device engineers may prefer to pay more attention towards the gray region of the graph which suggests the current saturation region for applied gate voltage.
Mosfet Threshold Voltage Equation
It reveals the current data that has touched the saturation point or the saturation limit. At this point, if the VDS is increased will result in a marginal increase in the current, but a small increase in drain current may lead to a much larger VDS.
For increased gate voltage levels, which enable the MOSFET to turn ON fully, the green shaded area will show us the operating point for the process, indicated as resistive (or Ohmic) region.
Please note that the curves here show the typical values only, and does not include any minimum or maximum boundaries.
While operating at lower ambient temperatures, the device will require higher gate voltage to stay in the resistive region, which may go upward at the rate of 0.3 %/°C.
What is MOSFET RDS(on)
When device engineers have to encounter the output characteristics of the MOSFET, they will essentially want to learn about the RDS (on) of the device with reference to the specific operating conditions.
Generally, this can be a mix of VGS and IDS across the area where the curve has deviated from the straight line into the portion indicated by the gray shade.
Considering the example discussed above, a gate voltage of 3.1 V with an initial current of 10 Amps, the engineers will know that the RDS(on) will tend to be greater than the estimated value. Having said this, do we expect the MOSFET manufacturer to furnish an approximate data regarding this?
With both the quantities VDS and IDS readily obtainable in the curve it may become too enticing, and is often surrendered into, to divide the two quantities at the resultant RDS(on).
However, sadly we do not have an RDS(on) for the assessment here. It seems to be unavailable for the mentioned situations since for any section of the load line representing a resistance has to cross through the origin in a linear manner.
That said, it may be possible to simulate the load line in an aggregated form like a non-linear resistance.
At the minimum, this will guarantee that any understanding of practical working is sustained at the origin (0, 0).
Gate Charge Curve Characteristics
It is the gate charge curve data that actually gives us a real hint regarding the turn ON specs of the MOSFET as shown in the figure below:
Although the above curve is a standard inclusion in all MOSFET datasheets, the underlying indications are seldom comprehended by the MOSFET user.
Moreover, the modern advancement in the MOSFET layouts, such as trench and shielded gates, call for a revised addressing of the data.
For example, the specification named 'gate-charge' may appear slightly misleading by itself.
The linear and divided sections of the curve do not appear like voltage charging a capacitor, regardless of how much non-linear value it may exhibit.
To be precise, the gate charge curve signifies an associated data of two non parallel capacitors, having dissimilar magnitudes and carrying different voltage levels.
In theory, the functional capacitance as witnessed from MOSFET gate terminal is defined with the equation:
Ciss = Cgs + Cgd
where Ciss = gate capacitance, Cgs = gate source capacitance, Cgd = gate drain capacitance
Although it may appear rather simple to measure this unit and specify in the datasheets, it must be noted that the term Ciss is actually not a real capacitance.
It may be completely wrong to think that a MOSFET is turned ON merely through a voltage applied on the 'the gate capacitance Ciss'.
As indicated in the above figure, just before a MOFET turns ON, the gate capacitance has no charge, but the capacitance at gate-drain Cgd possesses a negative charge which needs to be eliminated.
Both these capacitance have a non-linear nature and their values largely vary as the applied voltages vary.
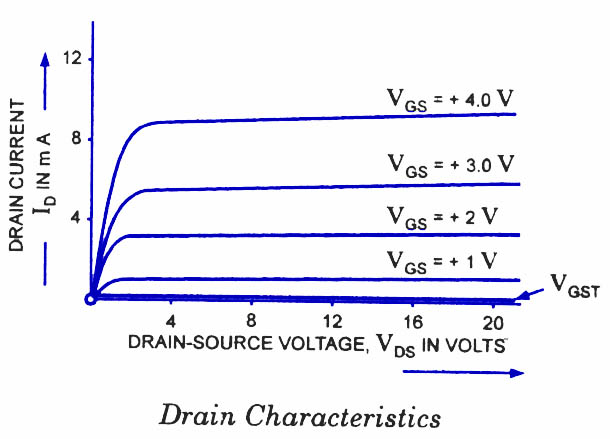
Hence, it is important to note that it is the stored charges of the MOSFET that determines its switching characteristics, and not the capacitance value for a specific voltage level.
Since the two capacitance elements constituting Ciss have different physical attributes, they tend to get charged with dissimilar voltage levels, requiring the turn ON process of the MOSFET also to go through two stages.
The precise sequence may be different for resistive and inductive applications, but typically most practical loads being highly inductive, the process could be simulated as depicted in the following figure:
Gate Charge Timing Sequence
The gate charge timing sequences of the MOSFET can be studied from the diagram below:
It may be understood with the following explanation:
- T0 - T1: Cgs charges from zero to VGS(th). VDS or IDS does not go through any changes.
- T1-T2, current starts rising in the MOSFET in response to the increasing gate voltage from VGS(th) upto the plateau voltage Vgp.
- Here, IDS increases and reaches to full load current from 0 V, although VDS remains unaffected and constant. The associated charge is formed through the integral of Cgs from 0 V to Vgp, and Qgs given in the datasheets.
- T2 - T3: Observe the flat region between T2 and T3, it's called the Miller plateau.
- Before the switch ON, Cgd charges and holds up to the supply voltage VIN, until IDS reaches the peak value I(load) at T2.
- The time between period T2 and T3, the negative charge (VIN - Vgp) gets converted into positive charge with respect to the plateau voltage Vgp.
- This can be also visualized as the falling of the drain voltage from VIN to around almost zero.
- The charge involved is equal to around the Cgd integral from 0 to Vin, which is shown as Qgd in datasheets.
- During T3 - T4, the gate voltage climbs from Vgp to VGS, and here we find hardly any change for VDS and IDS, but the effectual RDS(on) drops slightly as the gate voltage rises. At some voltage level above Vgp, provides the manufactures enough confidence to fix the upper limit on the effective RDS(on).
For Inductive Loads
The rise of current in MOSFET channel due to an inductive load needs to be completed before the voltage starts falling.
At the start of the plateau, the MOSFET is in the OFF state, in the presence of a high current and voltage across drain to source.

Hence, it is important to note that it is the stored charges of the MOSFET that determines its switching characteristics, and not the capacitance value for a specific voltage level.
Since the two capacitance elements constituting Ciss have different physical attributes, they tend to get charged with dissimilar voltage levels, requiring the turn ON process of the MOSFET also to go through two stages.
The precise sequence may be different for resistive and inductive applications, but typically most practical loads being highly inductive, the process could be simulated as depicted in the following figure:
Gate Charge Timing Sequence
The gate charge timing sequences of the MOSFET can be studied from the diagram below:
It may be understood with the following explanation:
- T0 - T1: Cgs charges from zero to VGS(th). VDS or IDS does not go through any changes.
- T1-T2, current starts rising in the MOSFET in response to the increasing gate voltage from VGS(th) upto the plateau voltage Vgp.
- Here, IDS increases and reaches to full load current from 0 V, although VDS remains unaffected and constant. The associated charge is formed through the integral of Cgs from 0 V to Vgp, and Qgs given in the datasheets.
- T2 - T3: Observe the flat region between T2 and T3, it's called the Miller plateau.
- Before the switch ON, Cgd charges and holds up to the supply voltage VIN, until IDS reaches the peak value I(load) at T2.
- The time between period T2 and T3, the negative charge (VIN - Vgp) gets converted into positive charge with respect to the plateau voltage Vgp.
- This can be also visualized as the falling of the drain voltage from VIN to around almost zero.
- The charge involved is equal to around the Cgd integral from 0 to Vin, which is shown as Qgd in datasheets.
- During T3 - T4, the gate voltage climbs from Vgp to VGS, and here we find hardly any change for VDS and IDS, but the effectual RDS(on) drops slightly as the gate voltage rises. At some voltage level above Vgp, provides the manufactures enough confidence to fix the upper limit on the effective RDS(on).
For Inductive Loads
The rise of current in MOSFET channel due to an inductive load needs to be completed before the voltage starts falling.
At the start of the plateau, the MOSFET is in the OFF state, in the presence of a high current and voltage across drain to source.
Between the time T2 and T3, a charge Qgd is applied to the gate of the MOSFET, wherein the MOSFET characteristic transforms from constant current to constant resistance mode at the end.
When the above transition happens, no noticeable change in the gate voltage Vgp takes place.
This is the reason it is never a wise idea to relate a MOSFET turn ON process with any particular level of gate voltage.
Fashion design flat sketch pc. The same may be true for the switch OFF process, which demands the same two charges (discussed earlier) to be eliminated from the gate of the MOSFET in the opposite order.
MOSFET Switching Speed
While Qgs plus Qgd together ensures that the MOSFET will switch ON fully, it does not tell us about how quickly this will happen.
How fast the current or voltage will switch is decided by the rate through which the charge elements at the gate are applied or removed. This is also termed as the gate drive current.
Although a fast rise and fall rate ensures lower switching losses in MOSFETs, these may also give rise to system level complications related to increased peak voltages, oscillations, and electromagnetic interference, especially during the turn off instants of the inductive load.
The linearly falling voltage depicted in the above Fig.7 manages to take a constant value of Cgd, which may hardly happen to MOSFETs in practical applications.
To be precise, the gate-drain charge Cgd for a high voltage super junction MOSFET such as SiHF35N60E exhibits a significantly high linear response, as can be seen the following figure:
The variation range that exists in the value of Crss (reverse transfer) is more than 200:1 within the initial 100 V. Due to this the actual fall time of voltage against the gate charge curve appears more like the dashed line shown in red color in figure 7.
At higher voltages, the rise and fall times of the charges, along with their equivalent dV/dt values are more reliant on the value of Crss, instead of the integral of the whole curve indicated as Qgd.
When users want to compare MOSFET specs within different design environments, they should realize that MOSFET with half the Qgd value won't necessarily feature two times faster switching rate, or 50% less switching losses.
This is because, according to the Cgd curve and its magnitude at higher voltages, it may be quite possible for a MOSFET to have a low Qgd in datasheet, but without any increase in the switching speed.
Summarizing
In actual implementation, the turning ON of a MOSFET happens through a series of processes, and not with a predetermined parameter.
Circuit designers must stop imagining that VGS(th), or voltage levels could be used as the gate voltage for switching the MOSFET output from high to low RDS(on).
It may be futile to think about having an RDS(on) below or above a specific gate voltage level, since gate voltage level doesn't intrinsically decide the turn ON of a MOSFET. Rather it's the charges Qgs and Qgd introduced into the MOSFET that execute the job.
Humana copay colonoscopy. You may find the gate voltage rising above VGS(th) and Vgp during the charge/discharge process but these are not so important.
Fet Threshold Voltage
Likewise, how fast todays MOSFET may turn ON or OFF can be a complex function of Qgs or Qgd.
Fet Threshold Voltage Chart
For evaluating MOSFET switching speeds, especially the advanced MOSFETs, the designer must go through a comprehensive study regarding the gate charge curve and the capacitance characteristic of the device.
Reference: https://www.vishay.com/
Threshold voltage is the point at which an electrical device is set to activate any one of its operations. This normally occurs within a transistor that continually monitors the power source for changes, ignoring those that are faint or inadvertently leaked through the system. Once the charge of incoming electricity is sufficient to meet the preset standard, the threshold voltage is met and power is allowed to flow throughout the device in order to enable it. Anything below the predefined threshold is contained and treated as a phantom charge.
Fet Low Gate Threshold Voltage
Although determining the threshold voltage in a device with a single circuit may seem relatively simple and straightforward, modern electronics require a fairly complex mathematical formula to set and regulate the various thresholds. An appliance like a dishwasher, for example, may be programmed to complete 20 or more functions dependent on the user's everyday requirements, and each separate phase that it enters is activated by an electrical charge. These subtle changes in power allow the device to know when to add more water, when to activate the drying mechanism, or how quickly to rotate the cleaning jets. Each of these activities is set to a separate threshold voltage, so when a number of elements needs to be activated at once, it requires a great deal of planning to ensure proper operation. The equation for calculating threshold voltage is the sum of the static voltage, plus twice the bulk potential and the voltage across the oxide.
A threshold voltage is normally constructed with a thin inversion layer that separates the insulating and the actual body of a transistor. Tiny holes that are positively-charged cover the surface of this region, and when electricity is applied, the particles within these voids are repelled. Once the current within both the inner and outer regions is equalized, the transponder allows a release of the energy to complete the circuit that activates the process. This entire process is completed within milliseconds, and the transistor constantly rechecks to ensure that the flowing current is justified, sapping the power once it is not.
What Is Threshold Voltage
Another term that is used when talking about transponders is metal oxide semiconductor field effect transistor (MOSFET) threshold voltage. These conductive switches are designed with either positive or negative charges much like in the above example, and they are the most common type of transistor within analog or digital devices. MOSFET transistors were originally proposed in 1925 and constructed in aluminum up until the 1970s, when silicon was discovered as a more viable alternative.